Carbon Dual-Cure Materials
Abstract
The required properties of a 3D-printed part depend on its intended use. For example, single-cure materials are suitable for many dental and prototyping applications, but fall short for demanding end-use applications across a wide range of industries. To solve this issue, Carbon developed dual-cure materials for the Carbon Digital Light Synthesis™ (Carbon DLS™) process that provide key advantages over single-cure resins, especially with respect to durability and other critical properties. Carbon’s dual-cure materials greatly expand the portfolio of available chemistries by blending UV-curable and thermally-curable chemistries into a single resin. Light activates the UV-curable chemistry during printing to first define the shape of the part, followed by a secondary baking step that activates the thermally curable components. This approach creates an interpenetrating polymer network in the final part, which can withstand the repeated stresses required of engineering-grade parts. Here we describe the dual-cure process in detail and compare it against the landscape of other 3D printing materials. When comparing dual-cure materials to competing single-cure materials targeted for similar uses, dual-cure materials outperform in several key attributes.
Problem Statement
Several 3D printing technologies including stereolithography (SLA), digital light processing (DLP), and jetting methods use photopolymer resins to generate parts. These resins and components are historically derived from the coatings and 2D-printing industries and are limited to a relatively small range of chemical functional groups (e.g., acrylates and methacrylates) that, when used in combination with various photoinitiators, are curable by light. In this paper, we refer to such resins as “single-cure,” in that exposure to light, and the subsequent reactions that occur, are the sole mechanism of polymerization. This holds true even when parts are exposed to UV-light post-printing in so-called “flood curing” processes which further propagate the same chemical reactions initiated during printing.
While single-cure resins are suitable for prototyping or generating simple custom-fit parts like dental models, they often lack the mechanical properties and durability required for production-grade parts, in competition with injection-molded thermoplastics or foams.
Other 3D printing technologies use the same thermoplastic materials used in injection molding such as nylon, acrylonitrile butadiene styrene (ABS), or poly(lactic acid) (PLA). While this offers mechanical property improvements compared to most single-cure UV-curable resins, the processing of thermoplastics using these printing technologies has intrinsic disadvantages—mainly feature resolution, porosity, and anisotropic (direction-dependent) mechanical properties. For example, the resolution of fused deposition modeling (FDM) is limited ultimately by the nozzle diameter of the extrusion process, which results in challenges for fine features. Selective laser sintering (SLS) results in porous parts that are prone to warpage. Additionally, the resolution of SLS is limited to the size of the powder particles (typically around 200 microns). In contrast, liquid, UV-curable resins used in inkjet, SLA, or DLP offer feature resolution comparable to injection-molded products but lack the requisite mechanical properties for many applications.
This intrinsic tradeoff between feature resolution/part accuracy and mechanical properties led Carbon to innovate a new approach. Could the speed of printing and fine feature resolution be attainable with UV-curable materials combined with the mechanical strength of thermally-curable materials, replacing injection molded parts with quality 3D-printed parts?
Background
Polymers are used in everything from pillows and insulation to bowling balls and medical devices. They are composed of repeating monomer units, which create long chemically-bonded molecular chains. The intrinsic properties of a polymeric material are dictated by several factors including:
- The chemical make-up of the polymer chains
- The length, or molecular weight, of the polymer chains
- The physical interactions between the chains
- The architecture of the chains (linear, branched, crosslinked, etc.)
- The morphology of the polymers (block, semi-crystalline, amorphous)
- Polymer processing and additives
All of these factors also play a critical role in polymer 3D printing processes and the properties of the resulting parts.
Polymerization
Polymer systems can be roughly divided into thermosets and thermoplastics. Thermosets, such as epoxy resins, silicone rubbers, or cyanate esters, typically come in the form of two-part reactive liquid precursors that are blended together before use. As these precursors react, polymer chains are formed and cross-linked together to form a chemically-bonded network. Once the curing reaction is complete, the thermoset generally cannot melt again when heated. Thermoset plastics typically have excellent thermal and chemical stability and are well-suited for applications like electronics and high-temperature composites.
In contrast, thermoplastics do not crosslink but instead rely on physical chain entanglements and polymer morphology for mechanical strength. As a result, thermoplastics can be melt-processed in operations like injection molding.
Both thermosets and thermoplastics are widely used in 3D printing processes. The resulting properties are a combination of both the intrinsic material properties and the processes used during printing and post-processing. Most 3D printing methods rely on a layer-by-layer process that results in poor entanglement or bonding of polymer chains at the interface between layers. This results in point defects at each layer and anisotropic mechanical properties. In contrast, Carbon Digital Light Synthesis (DLS) projects light through an oxygen-permeable window into a reservoir of UV-curable resin. As a sequence of UV images is projected, the part solidifies and the build platform rises. Just above the window, a dead zone prevents resin from adhering, which maintains a space for liquid resin to flow into the build area. This process creates a gradient of polymerization within each layer that significantly improves layer-to-layer adhesion, resulting in parts with isotropic material properties. Additionally, the reduction in adhesion forces allows for significantly faster printing.
Single-cure and its limitations
UV-curable resins have been in use in 3D printing for over 30 years. As stated previously, these resins use monomers and oligomers that originated in the inks, coatings, and adhesives industries. They are comprised of three main types of UV-reactive components, sometimes used in combination: vinyl-containing monomers (acrylate, methacrylate, etc.), thiol-ene, and cationic.
- Vinyl-containing components (e.g., acrylate, methacrylate) cure rapidly and are the most common type of single-cure resin used in photopolymerization technologies. They have good stability, decent mechanical properties, and come in a wide variety of functionalities. Parts made from these components are suitable for some applications, especially prototyping or dental appliances, but often have high shrinkage which can result in part warpage. Additionally, the range of mechanical properties is limited for these systems.
- Thiol-ene components are relatively new to 3D printing and use a “click chemistry” reaction between a thiol and an alkene to form a thioether. They form homogeneous networks via step-growth kinetics with flexible thioether linkages. This can result in a softer material with low modulus in the end product. They cure a bit slower (compared to vinyl-containing components) but yield decent properties with less shrinkage and warpage. However, these components are in limited supply, and they are also pungent, which can be problematic for end-use parts.
- Cationic components cure very slowly using a chain growth mechanism with a high number of cross-linking points along the polymer backbone. This slower process results in reduced shrinkage but typically brittle properties.
Most of these “single-cure” materials have two steps to their curing process: a UV-cure during the printing process and a UV post-cure, which is typically done in a curing chamber with a high-power UV source. In a typical case, the material undergoes partial polymerization in the printer and further conversion in the UV post-cure. The addition of heat during the UV post-cure, or heat generated from the post-cure itself, is often important to obtain high conversion and the best possible properties. Because the UV post-cure drives final properties, the “line-of-sight” of the light source is important. Internal sections or geometries that lead to “shadowing” are unlikely to reach full conversion and therefore may be weaker than the rest of the part. Non-uniform curing throughout a part is a significant limitation of this approach (Figure 1).
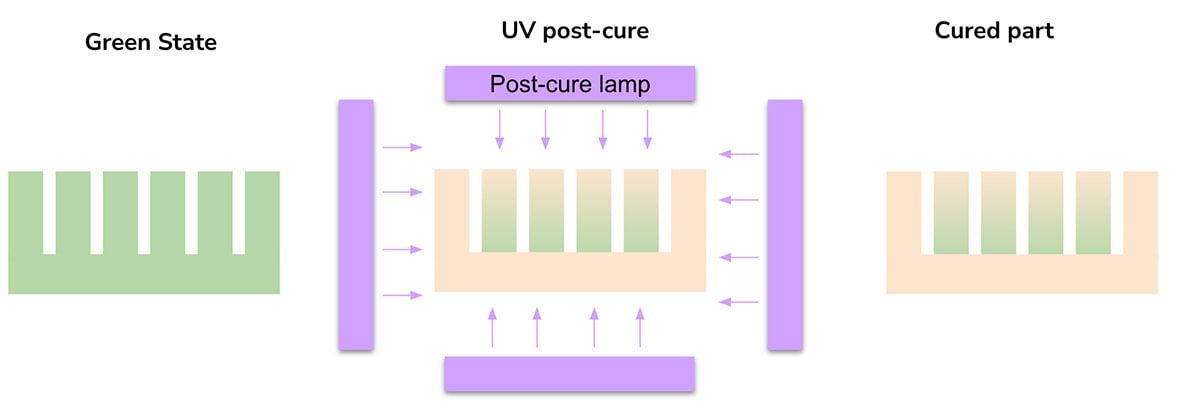
There are many uses for single-cure materials, especially in prototyping and oral health products. While the materials are useful in these contexts, they often lack the strength, toughness, and durability needed for production-grade parts used in more demanding applications such as footwear, automotive, and other industrial applications.
“Hybrid” Resins
Other UV-curing resins use two orthogonal photopolymer networks: vinyl free radical and cationic, commonly referred to as “hybrid” resins. While this involves two unique reactions, they are both UV-initiated reactions. One cures fast (using a methacrylate network) during the print to set the green state, and the second cures later, much slower and longer (using a cationic network). These types of resins offer advantages over single-cure resins, especially in terms of accuracy in stereolithography systems, but are still subject to the intrinsic limitations of photopolymers described earlier.
Solution
The required properties for a 3D-printed material depend on the intended use of the final part. For example, parts used in automotive applications need to withstand high temperatures while maintaining toughness, while materials used in medical devices must be biocompatible and tolerate sterilization processes (e.g., steam autoclave). Within elastomers, helmet padding should absorb energy while running sneaker midsoles should return energy to maximize running economy. Single-cure materials lack both the mechanical properties for demanding applications as well as the versatility of chemistries required for such a wide range of applications.
To address these challenges, Carbon leverages dual-cure chemistry to both expand significantly the palette of available chemistries for formulation, as well as incorporate orthogonal, thermally-cured polymers that are well suited for demanding applications. Dual-cure materials greatly expand the scope of formulation possibilities by combining UV-curable components with thermally-curable components such as those used in polyurethanes, epoxies, silicones, cyanate esters, and several other polymer systems.
Carbon DLS™ Delivers Attributes not Available from Traditional Polymer technologies
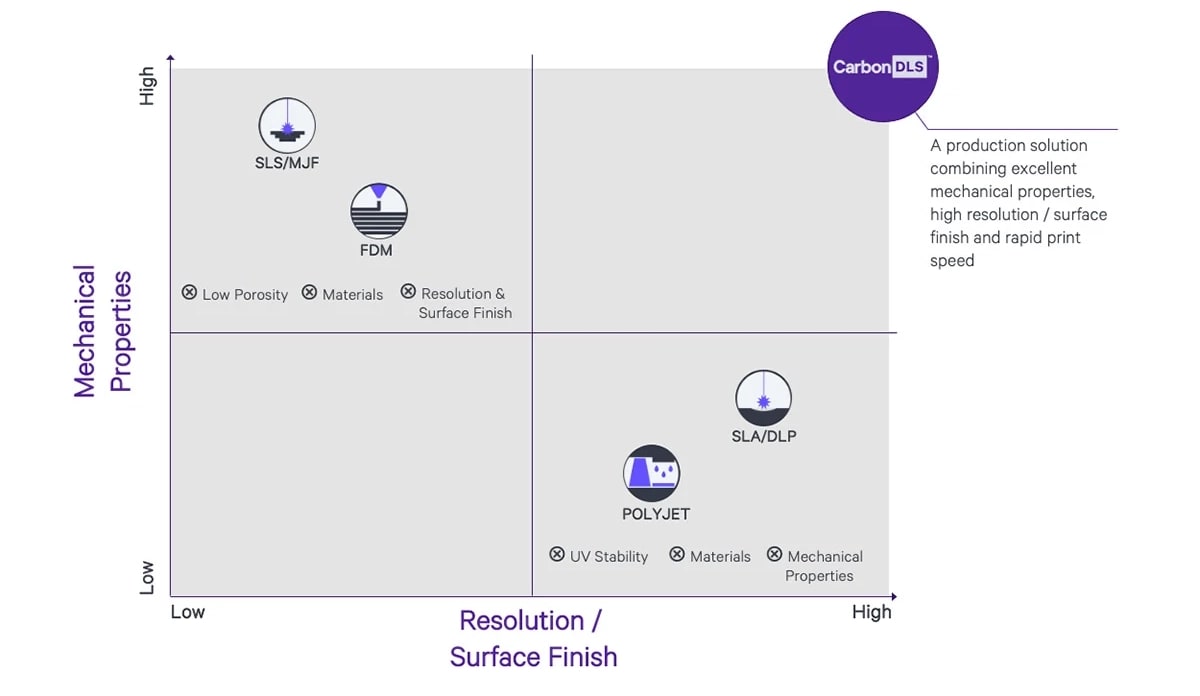
Carbon Dual-Cure and How it Works
Carbon’s dual-cure materials contain both UV-curable components, which are activated during printing, and thermally-curable components, which are activated in a secondary thermal bake. The inclusion of thermally-curable components significantly expands the formulation space and allows Carbon to build formulations with components commonly used in a variety of industries (e.g., epoxy/amine thermosets similar to those in carbon fiber prepreg; polyurethane prepolymers similar to those in foams for running sneakers). This broader chemical toolbox and uniform curing from heat drive the unique properties that can be achieved by dual-cure.
In one example, the UV-curable “Part A” contains a polyurethane prepolymer with reactive diluents and a thermal-curable “Part B” with diamine chain extenders. Prior to printing, these two parts are mixed together, with Part B solubilized into the first component. During printing, UV light solidifies the initial shape with the Part A material, forming a polymer scaffold, trapping the Part B material within that network. After the excess resin is cleaned from the “green” part, it is heated in an oven where the Part A network reacts with the Part B to form high molecular weight polyurethane/urea polymers. Molecular weight is crucial to polymer performance. Above a certain molecular weight, the chains are long enough to entangle, which adds toughness and durability to the polymer chains and improved part performance in properties like impact strength, tear strength, and/or fatigue resistance.
Carbon’s dual-cure process can also lead to micro-phase separation, a critical property of many high-performance polymers. Within the same polymer chain, there can be repeat segments of different monomer types. These differing polymer segments want to separate on a macro level into their own phases (like oil and water) but they are chemically linked. As a result, they spontaneously form nano-sized domains within the macroscopic matrix. For example, in elastomers (like EPU 41), hard polyurethane segments will stick together in high glass transition temperature (Tg) domains while the rest of the space is a low Tg soft segment. When stretched, the hard segments hold everything together. This separation adds fatigue resistance, impact strength, and toughness to the final product. The inverse is also true for rigid materials, like RPU 130. Nano-sized soft segments form impact-resistant domains throughout an otherwise rigid macroscopic matrix. This significantly improves the toughness of a material.
Examples using Carbon’s dual-cure materials
Every material has different strengths and weaknesses; what makes it useful is having the appropriate combination of properties for a particular application. In the following sections, we compare Carbon materials to traditional, single-cure photopolymer elastomeric materials. All values cited are current as of September 2022. This is presented to illustrate single-cure material limitations across the combination of mechanical properties, thermal properties, and durability.
End-Use, Energy-Returning Elastomer: EPU 41
Carbon’s first dual-cure resin created for a specific use was elastomeric polyurethane EPU 41. adidas needed a material for printed midsoles that had the requisite flexibility, durability, aesthetics, and energy return to meet or exceed existing foam midsole materials. Single-cure resins that might have the flexibility needed weren’t nearly durable enough to withstand the repeated compression from high-impact sports like running. Likewise, powder-based TPU materials did not have enough chain entanglement at the interface between particles, resulting in poor durability under repeat compression.
We first synthesized a custom polyurethane prepolymer as Part A, then added a photoinitiator and reactive diluents to lower the viscosity of the resin to make it compatible with printing. The diamine Part B is blended with Part A before use, and the initial UV-cured polymer network is formed during printing. After printing, the trapped Part B reacts with this prepolymer during the baking step, growing to high molecular weight polyurethane/ureas that phase separate to create highly resilient and tough elastomers. This route to high molecular weight polyurethanes is the key to EPU 41’s toughness and durability.
Below we compare EPU 41 and a single-cure material we will call “Single-Cure A” that also presents itself as being suitable for energy-returning, end-use applications like midsoles. While some mechanical properties of EPU 41 might be similar to Single-Cure A, Table 2 illustrates that the durability of EPU 41 is significantly better than Single-Cure A and therefore a much better choice of material for applications like midsoles.
Mechanical Properties
Material | Ultimate Tensile Strength (MPa) |
Elongation at Break (%) |
Shore A ASTM D2240 |
Tg (°C)
ASTM |
---|---|---|---|---|
Carbon EPU 41 | 8 | 250 | 72 | -10 |
Single-Cure A | 9 | 95 | 75 | 29 |
Table 1: Mechanical comparison of Carbon EPU 41 and Single-Cure A
Highlights:
- The Shore A hardness of the two materials is comparable, as measured by the ASTM D2240 durometer test. They are both in the 70s, indicating the hardness of the material is different only by a few degrees, and thus both are suited to a comparable application space.
- Both EPU 41 and Single-Cure A have comparable ultimate tensile strength, with Single-Cure A being slightly higher.
- The elongation at break of EPU 41 is more than double that of Single-Cure A.
- The glass transition temperature (Tg) of EPU 41 is lower by nearly 40°C, well below temperatures of most inhabited environments. This is critical for maintaining resilience across a range of use temperatures.
Additionally, EPU 41 passes biocompatibility for both in vitro cytotoxicity (ISO 10993-5) and skin irritation and sensitization (ISO 10993-10 and ISO 10993-23) when used with a specific workflow (see datasheet for details). Single-Cure A reports passing ISO 10993 and suggests contacting them for details. Similarly, as a production-grade material, EPU 41 is expected to encounter many potential chemicals. As a result, EPU 41 has detailed chemical compatibility for 16 common household cleaners, industrial fluids, and strong acid/base combinations. Single-Cure A has no reported testing regarding chemical compatibility.
Durability
Printed Name | Tear Strength (kN/m)ASTM D624 | Compression Set 23°C (%) ASTM D395-B | Bayshore Rebound Resilience (%) ASTM D2632 |
Rebound Resilience (%) ASTM D7121 |
Ross Flexing Fatigue (Notched) 23°C ASTM D1052 |
Ross Flexing Fatigue (Notched) -10°C ASTM D1052 |
---|---|---|---|---|---|---|
Carbon EPU 41 | 20 | 9 | n/a | -21 | >50,000 cycles | >40,000 cycles |
Single-Cure A | 3 | 30 | 30 | n/a | >20,000 cycles | >10,000 cycles |
Table 2: Durability comparison of Carbon EPU 41 and Single-Cure A
Highlights:
- In tear strength, EPU 41 is >6x that of Single-Cure A, as measured by ASTM D624.
- In flexing fatigue, EPU 41 has greater than 2x the performance at 23°C and approximately 4x the performance at -10°C, as measured by Ross flexing fatigue in ASTM D1052.
- In compression set testing, where lower scores indicate the material can better resist permanent deformation under a given temperature and deflection range, EPU 41 has 3x greater performance compared to Single-Cure A.
- EPU 41 and Single-Cure A use different testing methodologies to characterize rebound resilience. As a result, they are not directly comparable.
Additionally, for production-grade polymers, Carbon publishes detailed dynamic mechanical analysis (DMA) in technical datasheets, including for EPU 41. Single-Cure A does not publish any detailed DMA information.
End-Use, High Temperature, High Impact Rigid: RPU 130
Rigid polyurethane RPU 130 was designed to match the temperature resistance and impact toughness exhibited by unfilled thermoplastics, to enable use in consumer housings and automotive interior parts. This material uses the same blocked polyurethane prepolymer approach as EPU 41, but with different building blocks that yield a semi-rigid, tough, heat-resistant end part (rather than an elastomer).
The following sections compare RPU 130 with another single-cure, semi-rigid material focused on end-use parts, which is referred to as “Single-Cure B.” Single-Cure B has similar mechanical properties to RPU 130, but a significantly lower HDT that precludes use in industrial components or consumer goods that may be exposed to high temperatures. Note that this competitor’s datasheet reports values as a range, with a mean ± a value. They use the mean value to characterize the material, and the comparisons that follow use that same convention.
Mechanical Properties
Printed Name | Tensile Strength (MPa) | Tensile Modulus (GPa) | Elongation at Break (%) | Notched Izod 23°C (J/m)
ASTM D256 |
Shore Hardness (D)
ASTM D2240 |
HDT 0.455 MPa
ASTM D648 |
---|---|---|---|---|---|---|
Carbon RPU 130 | 35 | 1.0 | 100 | 75 | 77 | 120 |
Single-Cure B | 35 | 1.3 | 100 | 62 | 80 | 52.8 |
Table 3: Mechanical Comparison of Carbon RPU 130 and Single-Cure B
Both RPU 130 and Single-Cure B are semi-rigid materials, with lower strength, modulus, and hardness than purely rigid materials.
- In tensile strength, RPU 130 and Single-Cure B are comparable.
- RPU 130 has ~27% lower tensile modulus than Single-Cure B.
- In elongation at break, RPU 130 and Single-Cure B are comparable.
- In notched impact testing, RPU 130 has greater than 20% higher impact resistance, as measured by ASTM D256.
- RPU 130 has a greater than 120% higher heat deflection temperature at 0.455 MPa, as measured by ASTM D648.
As a production-grade material, Carbon also offers additional, important end-use testing data.
- RPU 130 passes flammability testing per FMVSS 302.
- Carbon publishes representative tensile and flex curves for RPU 130.
- Creep behavior shows RPU 130 can withstand 1.8 MPa of applied load compared at 65°C with only 1% strain over 700 minutes.
- RPU 130 has published mass gain for sunscreen (5-15%), engine oil (<5%), transmission fluid (<5%), and diesel (15-30%).
- RPU 130 has passed biocompatibility testing for in vitro cytotoxicity per ISO 10993-5 and testing for irritation and skin sensitization per ISO 10993-10 and ISO 10993-23 for a specific workflow (see datasheet for details).
Single-Cure B has no published, comparable testing available.
Durability
As a production-grade material, RPU 130 prioritizes durability and publishes significant information related to UV aging, thermal aging, environmental aging, isotropy, and dynamic mechanical analysis.
- After 400 hours of UV aging per ASTM D4459, RPU 130 retains:
- 40% of its elongation at break
- >80% of its ultimate tensile strength
- ~100% of its modulus
- >100% of its yield strength
- After 800 hours of thermal aging at 40° C, RPU 130 retains between 95 and 105% of its modulus, notched impact resistance, ultimate tensile strength, yield strength, and modulus (see datasheet for specifics).
- Additional testing is available at 85 and 105 °C.
Beyond the performance data listed,Single-Cure B offers little insight into its durability.
End-Use, High Temperature, High Functional Toughness Rigid: EPX 82
Thermally-cured epoxies are notably used as the matrix phase of high-performance glass and carbon fiber composites. These epoxies are strong, tough, and durable, but do not cure with UV light. EPX 82 contains a thermally-cured epoxy and aromatic diamine hardener along with UV-curable components, allowing it to be printed as a dual-cure resin with final part properties similar to 20% glass-filled PBT. It is thermally stable, chemically stable, and has good electrical properties. Notably, due to the specific thermally-cured epoxy/hardener component, EPX 82 has the highest relative thermal index (RTI) in the UL Blue Card photopolymer list at 90°C. These properties are useful in automotive and other industrial applications.
To illustrate how dual-cure chemistry provides advantages over single-cure, we will contrast this with another commercially-available, high-stiffness, high-temperature, and functional-toughness material that we will refer to as “Single-Cure C.” The following sections show that EPX 82 and Single-Cure C prove to have some similar properties, but EPX 82 has the impact resistance and durability properties required for applications like electrical connectors.
Mechanical Properties
Printed Name | Tensile Strength (MPa) | Tensile Modulus (GPa) | Elongation at Break (%) | Unotched Izod 23°C (J/m) ASTM D4812 |
Notched Izod 23°C (J/m) ASTM D256 |
Shore Hardness (D) ASTM D2240 |
HDT 0.455 MPa ASTM D648 |
---|---|---|---|---|---|---|---|
Carbon EPX 82 | 80 | 2.8 | 5 | 370 | 45 | 89 | 130 |
Single-Cure C | 89 | 3.2 | 6 | – | 22 | 89 | 120 |
Table 4: Mechanical properties comparison of EPX 82 and Single-Cure C
Highlights
- Both materials offer comparable properties in tensile strength, tensile modulus, and elongation at break, with Single-Cure C scoring slightly higher in each.
- EPX 82 has more than double the impact resistance of Single-Cure C, as measured by ASTM D256. Additionally, EPX 82 publishes unnotched IZOD impact testing.
- EPX 82 leads Single-Cure C in heat deflection temperature by 8%, as measured by ASTM D648.
As a production-grade material, for EPX 82 Carbon offers additional, important end-use testing data. Single-Cure C does not report on any of these attributes.
- EPX 82 is tested for mass gain with 16 different household chemicals, industrial fluids, and strong acid/alcohol/bases, posting <5% gain for all except ethanol, which is between 5–15% gain. Single-Cure C does not report any chemical compatibility.
- EPX 82 passes USCAR2 chemical compatibility testing. Single-Cure C reports no chemical compatibility.
- EPX 82 passes biocompatibility testing for in vitro cytotoxicity per ISO 10993-5 and for irritation and skin sensitization per ISO 10993-10 and ISO 10993-23 for a specific workflow (see datasheet for details).
- EPX 82 passes stringent vehicle interior air quality benchmarks including: odor (VDA 270), volatile organics (VOC) and semi-volatile organics (VDA 278), and fogging (DIN 75201, Method B, Gravimetric).
Durability
As a production-grade material, EPX 82 prioritizes durability and publishes significant information related to UV aging, thermal aging, thermal shock, dynamic mechanical analysis, conditioned performance after water uptake, and specific automotive connector-related aging and cycling tests.
Highlights
- After 400 hours of UV aging per ASTM D4459, EPX 82 retains:
- 20% of its elongation at break
- ~100% of its modulus
- >80% of its yield strength
- EPX 82 takes up approximately 2% by weight of water at 23 °C and 50% relative
- humidity in equilibrium conditions.
- EPX 82 retains between 75% and 110% of its initial material properties after 1008 hours of heat aging at 125 °C.
- EPX 82 retains between 90% and 100% of its initial material properties after 40 cycles of 8-hour, joint thermal and humidity cycling.
- EPX 82 retains between 80% and 105% of its initial material properties after 100 cycles of thermal shock testing from -40 °C to 125 °C.
Beyond the property data listed before, Single-Cure C offers little insight into its durability in intended applications.
Conclusion
Single-cure resins are widely used in prototyping applications and a variety of lower-requirement 3D-printed parts such as dental or manufacturing aids. Many applications, however, require engineering-grade performance that single-cure resins, to date, have failed to meet. In contrast, dual-cure materials are specifically designed for high-performance characteristics like heat resistance, long-term thermal stability, impact toughness, and durability to repeated stresses. In their relatively short history, dual-cure resins have established entirely new markets for 3D-printed end-use parts. Examples include elastomer lattices for footwear and protective padding, as well as production-grade automotive parts such as electrical connectors and other interior components. The broader material palette enabled by this approach allows for the creation of materials that better match the performance of traditional manufacturing polymers, and opens the door to completely novel property combinations.
3D as It’s Meant to Be
Interested in utilizing Carbon to accelerate product development? Reach out to us at sales@carbon3d.com to learn more!